Published: Jan 02, 2025
We engineered split group I introns for programmable, predictable, orthogonal, and modular gene expression regulation and integrated them with protein splicing for single-layer multi-input logic computation. Our findings will unleash the signal-processing capabilities of a single regulatory gene for scaling up genetic circuits.
Programming genetic circuits to control cellular behavior as easily as programming electronic circuits has been the ambition of synthetic biology since its birth in the 2000s. Guided by electronic engineering principles, synthetic biologists have programmed bacteria and eukaryotic cells to execute various functions, such as logic computation, oscillation, and memory [1]. However, the synthetic genetic circuits we can build are far too simple compared to the endogenous genetic networks.
What limits the complexity and scale of synthetic genetic circuits? Our research group, supervised by Prof. Baojun Wang (https://www.wanglab.net/), has been pursuing the answer to this question. We have explored various mechanisms and strategies to build genetic circuits, including transcription factor cascades [2], DNA sponge [3], CRISPR activation system [4, 5], and split intein [6, 7].
Then we realized two key factors — 'building block' and 'design strategy' — limited the scaling of genetic circuits. In this recently published paper, we addressed these two challenges by creating novel building blocks based on RNA trans-splicing and proposing a novel genetic circuit design strategy by coupling RNA and protein splicing.
Creating new building blocks
Genetic circuits are built on a set of genetic elements (biological parts), such as promoters, terminators, and ribosome binding sites (RBS). Therefore, implementing complex genetic circuits requires many high-quality biological parts that are currently lacking. We turned our eyes to RNA-level regulation to discover novel, high-quality biological parts, due to its high programmability, fast dynamics, and low metabolic burden.
Nature has evolved many mechanisms to regulate the activity of RNA molecules, such as cleavage, degradation, and splicing. Among these mechanisms, RNA splicing plays a critical role in the maturation of functional RNAs. During the RNA splicing process, a non-coding sequence, called intron, is removed from the precursor RNA, and the flanking coding sequences, called exons, are joined together to generate a mRNA that can be translated into proteins.
Although widely known to occur in eukaryotic cells, RNA splicing can also occur in bacteria when catalyzed by the group I intron. Group I introns are autocatalytic introns that naturally perform cis-splicing, excise themselves from precursor RNAs and join the flanking RNA sequences (5' and 3' exons) (Fig. 1a). The group I intron could be split into two halves, which would self-assemble to reconstitute an active intron structure for trans-splicing (Fig. 1b). The autocatalytic mechanism and inherent programmability of group I introns make them attractive to act as building blocks for genetic circuits.
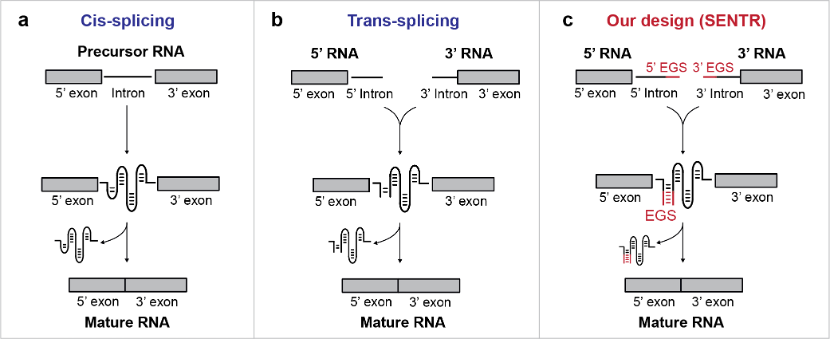
In this study, we developed a novel gene regulatory tool (named 'SENTR') based on split-intron-enabled RNA trans-splicing. We fused a pair of de-novo-designed RNA guides, named EGSs (External Guide Sequences), to the intron halves to regulate the intron assembly and control the trans-splicing activities (Fig. 1c). Therefore, we could alter the trans-splicing activities of a target mRNA by modifying the lengths and sequences of EGSs. We also used machine learning models to predict trans-splicing activities from the EGS sequences. Moreover, we could construct orthogonal RNA splicing pathways (pathways that function independently with each other) by designing EGSs with low sequence similarities.
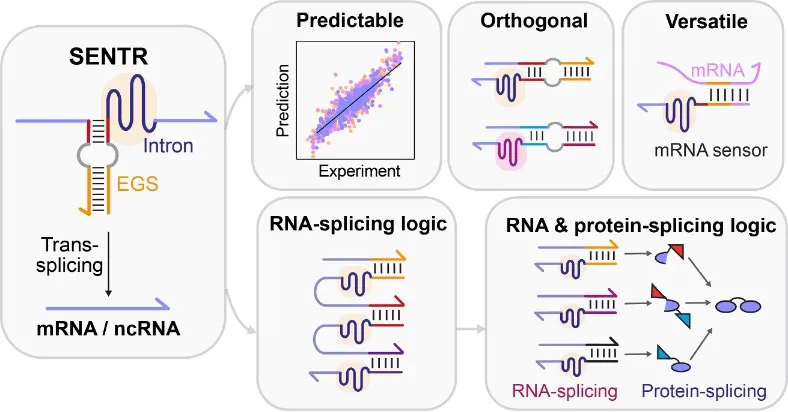
SENTR allowed versatile, modular and robust regulation of various genes (fluorescent proteins, transcription factors, and single-guide RNAs) (Fig. 2). Furthermore, the EGSs were very flexible and could be designed to hybridize with intracellular mRNAs and synthetic small RNAs. Leveraging the computationally designed interactions between SENTR and these RNAs, we realized the sensing of intracellular mRNAs of antibiotic-resistance genes and implementation of six two-input logic gates (AND, NAND, IMPLY, NIMPLY, OR, NOR).
Establishing the novel circuit design paradigm
Another key challenge in implementing complex genetic circuits is the over-consumption of transcription factors. For instance, building a four-input AND gate requires six transcription factors and chaperones in a conventional layered design [8]. In this study, we proposed a novel circuit design strategy by integrating RNA and protein splicing, allowing us to use a single transcription factor to build multi-input AND and NAND gates.
In this split intron and intein-enabled circuit design strategy, the input RNAs first underwent intron-catalyzed RNA splicing reactions to produce mRNAs; the translated peptides then underwent intein-catalyzed protein splicing reactions to produce functional transcription factors. Incorporating RNA and protein trans-splicing assimilates the input signals efficiently so that we could transform a single transcription factor into the four-input AND gate, four-input NAND gate, and six-input AND gate.
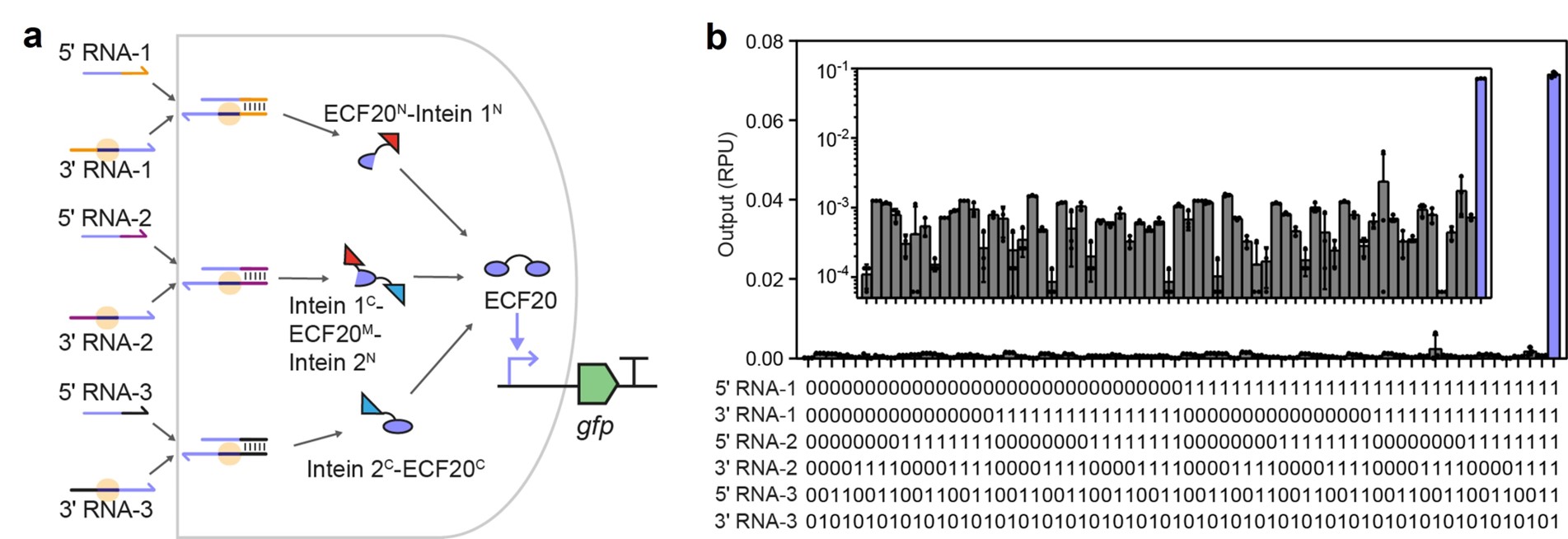
Take the six-input AND gate as an example. The six-input AND gate was constructed by inserting two orthogonal pairs of split intein and three orthogonal pairs of split introns into the ecf20 gene (Fig. 3). Once transcribed, the six input RNAs first underwent three RNA splicing reactions to produce three peptides, splicing into a functional transcription activator ECF20. The ECF20 could then recognize its cognate promoter to trigger the expression of the reporter gene. This six-input AND gate is the most complex AND circuit reported, consuming only one transcription factor. By comparison, the conventional layered design requires ten transcription factors to realize the same function8, meaning that our strategy increased the information processing capacity of a single regulator gene by tenfold.
We believe that our work will be of interest to the broad scientific community, in particular to synthetic and chemical biology researchers who seek new enabling tools and design strategies to scale up gene circuits, and to RNA biologists and biomedical researchers who seek methods to study and optimize ribozyme designs for fundamental research or clinical purposes.
Our paper:
Gao Y, Mardian R, Ma J, Li Y, French C and Wang B*, “Programmable trans-splicing riboregulators for complex cellular logic computation”, Nature Chemical Biology, 2025. doi
References
-
Gao, Y., Wang, L. & Wang, B. Customizing cellular signal processing by synthetic multi-level regulatory circuits. Nat. Commun. 14, 8415 (2023).
-
Wan, X. et al. Cascaded amplifying circuits enable ultrasensitive cellular sensors for toxic metals. Nat. Chem. Biol. 15, 540–548 (2019).
-
Wan, X., Pinto, F., Yu, L. & Wang, B. Synthetic protein-binding DNA sponge as a tool to tune gene expression and mitigate protein toxicity. Nat. Commun. 11, 5961 (2020).
-
Liu, Y., Wan, X. Y. & Wang, B. J. Engineered CRISPRa enables programmable eukaryote-like gene activation in bacteria. Nat commun 10, (2019).
-
Liu, Y. et al. Reprogrammed tracrRNAs enable repurposing of RNAs as crRNAs and sequence-specific RNA biosensors. Nat. Commun. 13, 1937 (2022).
-
Pinto, F., Thornton, E. L. & Wang, B. An expanded library of orthogonal split inteins enables modular multi-peptide assemblies. Nat. Commun. 11, 1529 (2020).
-
Ho, T. Y. H. et al. A systematic approach to inserting split inteins for Boolean logic gate engineering and basal activity reduction. Nat. Commun. 12, 2200 (2021).
-
Moon, T. S., Lou, C., Tamsir, A., Stanton, B. C. & Voigt, C. A. Genetic programs constructed from layered logic gates in single cells. Nature 491, 249–253 (2012).